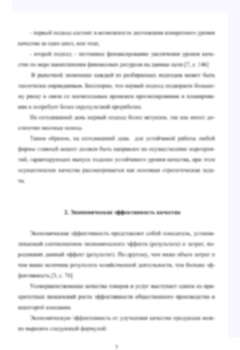
Производная группа Пикара самоинъективных алгебр конечного типа представления
В работе описаны производные группы Пикара симметрических алгебр конечного типа представления типа D.
1. Introduction 1
2. Preliminaries 2
3. Modified Brauer trees and mutations for type D 4
3.1. Algebras 5
3.2. Mutations 8
4. The two algebras Λ and R 14
4.1. The Picard group of Λ 15
4.2. Spherical twists on Λ 16
4.3. Some relations in TrPic(R) 20
5. Main result 22
6. Standard series of mutations 23
6.1. The Double Edge case 23
6.2. The Triple Tree case 24
7. Autoequivalences of the form (μ st )−1 ◦ μ+ ◦ μst 25 μ+j(G) j G
7.1. Double Edge type
7.2. The Triple Tree type
7.3. Finishing the proof
8. Appendix References
26 29 36 37 40
Derived categories were introduced by Verdier, a student of Grothendieck, in the 1960-s. Since then, they have not only transformed the landscape of algebraic geometry, becoming both an indispensable technical tool and one of the central objects of study, but also have spread to many other areas of mathematics and even physics. The passage from abelian categories to their derived categories or, in other words, from working with cohomology of complexes to working with complexes themselves, turned out to be a very fruitful idea. It allows to work around the problems occurring with non-exact functors between abelian categories and obtain new insights into numerous geometric and algebraic structures. It is no exaggeration to say that derived categories provide a unique way to connect mathematical objects of different nature. One of the first remarkable results demonstrating this phenomenon is the famous theorem stating that the derived category of quasi-coherent sheaves on the projective space Pn over a field is equivalent to the derived category of an explicitly described finite-dimensional algebra (see [6], [8]). Moreover, Bondal and Van den Bergh showed [9] that the derived category of quasi-coherent sheaves on any quasi-compact quasi-separated scheme over a field is equivalent to the derived category of some dg-algebra.
Nevertheless, despite many years of excessive research, derived categories in general remain rather difficult to tackle. It is often natural to study mysterious objects by understanding their symmetries or, more precisely, in our case, autoequivalences of derived categories. However, describing all autoequivalences of a derived category, or even constructing some non-trivial ones, is a difficult problem, because there are not many general principles to rely on.
Let k be an algebraically closed field and let A and B be k-algebras. The classical Morita theory studies the relation of Morita equivalence. Namely, A and B are Morita equivalent if their module categories are equivalent. One can go further and consider the relation of derived equivalence instead. We say that two algebras A and B are derived equivalent if their bounded derived categories Db(A) and Db(B) are equivalent as triangulated categories. Rickard and Keller [17], [18], [15] provided an answer to the question of when two algebras are derived equivalent using the language of tilting complexes. These discoveries became a major landmark in tilting theory and jumpstarted the study of equivalences of derived categories in algebra outside of algebraic geometry, at the same time helping understand the interplay between representation theory and algebraic geometry. The derived Picard group TrPic(A) of A, introduced independently by Rouquier-Zimmermann [21], and Yekuteli [27], is the group of so-called standard autoequivalences of the bounded derived category Db(A) modulo natural isomorphisms. Equivalently TrPic(A) can be defined as the group of isomorphism classes of two-sided tilting complexes over A with respect to the tensor product. The derived Picard groups is an important derived invariant of an algebra. Derived Picard groups are closely related to the Hochschild cohomology [16], another derived invariant of an algebra. They are also known to be locally algebraic [27].
1
The derived Picard group of an algebra is a natural analogue of the classical Picard group in the context of the derived Morita theory of Rickard and Keller. However, while the classical Morita theory for finite-dimensional k-algebras is very well understood (i.e. the classical Picard group is the group of outer automorphisms), there is no general recipe known for computing derived Picard groups. So far, complete descriptions of derived Picard groups have been obtained only for several very nice classes of algebras, e.g. for commutative, for local, for finite-dimensional hereditary algebras, for derived discrete algebras, for affine Azumaya algebras, for preprojective algebras of types A and D, and for self-injective Nakayama algebras (see [21], [23], [11], [25], [24], [29]). On the other hand, the study of derived Picard groups can not only contribute to the understanding of the algebras in question, but also shred some light on the structure of their derived categories. In particular, the problem of describing the derived Picard group of an algebra is closely related to the problem of classifying all tilting complexes in its derived category, hence it can give some insights into t−structures in this category.
Self-injective algebras play a prominent role in the representation theory of algebras. It is a wide class of algebras that includes many curious examples frequently appearing “in nature” (e.g. group algebras of finite groups, finite- dimensional Hopf algebras). At the same time, it is manageable enough to study, since self-injective algebras share quite a lot of non-trivial properties and structures (e.g. their stable categories are triangulated). The simplest ones among self-injective algebras are self-injective algebras of finite representation type. Such algebras were classified up to derived equivalence by Asashiba [5], namely, they correspond to triples (Γ,q,t), where Γ is a simply-laced Dynkin diagram, reflecting the form of the stable Auslander-Reiten quiver of an algebra, q is a rational number called frequency and t is a number called torsion (which is either 1, 2 or 3). For type A and torsion 1 the derived Picard groups of such algebras were described by Volkov and Zvonareva [30], [29].
In this paper we explicitly describe derived Picard groups of symmetric representation-finite algebras of type D. In terms of the classification we mention above, these are precisely the algebras of type D with frequency 1 and torsion 1. We prove that the derived Picard groups of such algebras are isomorphic to extensions of braid groups of type D by shifts and the classical Picard groups. More precisely, the result we obtain is as follows. Let m ≥ 4 and let BDm denote the Artin group of type Dm (the generalised braid group, see Definition 2.5). We denote the set of standard generators of the braid group BDm , i.e. we will always assume that σi satisfy braid relations of type Dm. Let c = σ1 …σm ∈ BDm. When we write κa, a ∈ k∗, we mean that κa satisfy the same relations which hold for the corresponding elements of the multiplicative group of the field.
Theorem (Theorem 6). Define the following group by generators and relations
G = σ ∈B ,{κ } ∗,τ,s τσi =σiτ (i̸=1,2); τσ1 =σ2τ; τ2 =e;
m i Dm a a∈k s and κa ∀a ∈ k∗ commute with every generator
Let Gm = G m/⟨cm−1s−2m+3κ−1⟩ if m is even and Gm = G m/⟨cm−1s−2m+3κ−1τ⟩ if m is odd. Then the
derived Picard group of any algebra of type (Dm,1,1) is isomorphic to Gm.
A result on the faithfulness of braid group actions [26] gives us a braid group of type D generated by spherical twists inside the derived Picard group. After that, the most difficult part is to show that every standard autoe- quivalence can be obtained as a composition of several spherical twists, modulo some obvious autoequivalences, i.e. those induced by outer automorphisms of the algebra and the shift. To do this we apply the technique used by Zvonareva for Brauer star algebras [30]. The key ingredient there is the result of Aihara [1] stating that every symmetric representation-finite algebra is tilting-connected. This allows, although not immediately, to reduce the problem to computing all sequences of silting mutations (in the sense of Aihara and Iyama [4]) of a particular form and then expressing them as compositions of twists, shifts and outer automorphisms (Sections 6-8 of the paper). In turn, for this we first need to develop an understanding of silting mutations for the class of algebras we are considering. We therefore start by introducing a combinatorial-geometric model for computing silting mutations in our case, generalising the classical concepts of Brauer trees and Kauer moves on them to suit our needs (Section 3 of the paper).
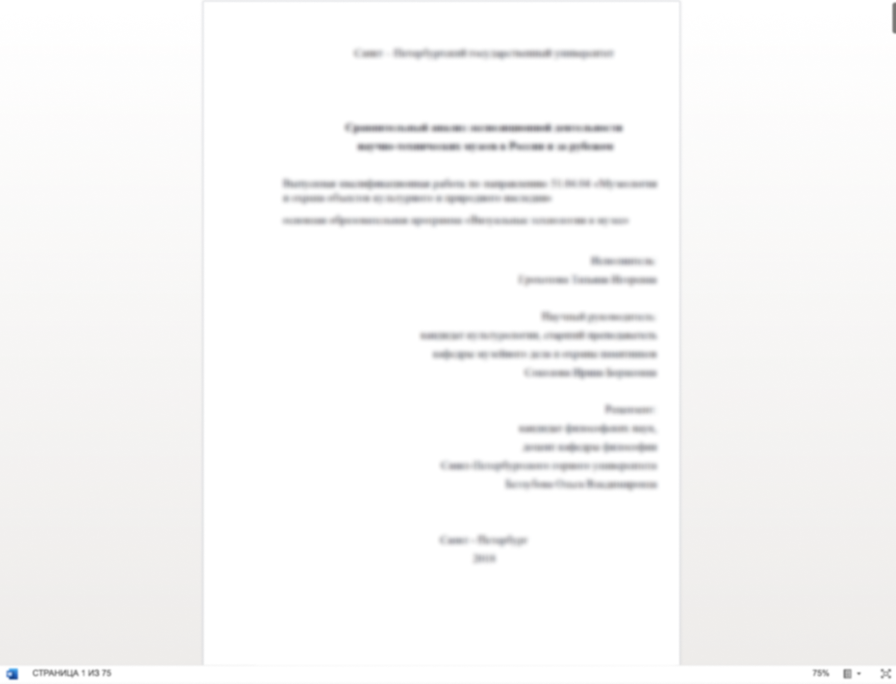
Хочешь уникальную работу?
Больше 3 000 экспертов уже готовы начать работу над твоим проектом!